Material efficiency in clean energy transitions
About this report
This analysis examines the potential for material efficiency and the resulting energy and emissions impact for key energy-intensive materials: steel, cement and aluminium. It includes deep dives on the buildings construction and vehicles value chains, and outlines key policy and stakeholder actions to improve material efficiency. Important actions include: increasing material use data collection and benchmarking; improving consideration of the life-cycle impact in climate regulations and at the design stage; and promoting repurposing, reuse and recycling at end of product and buildings lifetimes.
Highlights
Economic development has historically relied on increasing material demand, which has led to growing energy consumption and carbon dioxide (CO2) emissions from materials production. Applying material efficiency strategies throughout value chains can help to decouple these trends.
Clean energy transitions will affect established material demand trends. In the Clean Technology Scenario, material efficiency and technology shifts result in lower material demand relative to the Reference Technology Scenario, in which material demand trends broadly follow historical trends. By 2060, in the Clean Technology Scenario, material demand is lower than in the Reference Technology Scenario: 24% lower for steel, 15% lower for cement and 17% lower for aluminium. Material efficiency contributes approximately 30% of the combined CO2 emissions reduction for these three materials between the two scenarios in that year.
Considerable potential exists to push material efficiency even further than in the Clean Technology Scenario. Pursuing material efficiency to highly ambitious yet achievable limits in a Material Efficiency variant leads to additional demand reductions for steel (16%) and cement (9%) in 2060. Demand for aluminium increases slightly relative to the Clean Technology Scenario (by 5% in 2060), but CO2 emission benefits at other stages of the value chain outweigh this increase.
Material efficiency strategies result in more moderate deployment needs for low-carbon industrial process technologies to achieve the same decarbonisation outcome. In the Material Efficiency variant, cumulative industrial CO2 emissions are the same as in the Clean Technology Scenario, although the emissions intensity is higher for steel (by 4% in 2060) and cement (by 7% in 2060). The emissions intensity of aluminium is somewhat lower (by 9% in 2060). Combined cumulative capital investment on low-carbon industrial process technologies for steel, cement and aluminium is 4% lower by 2060 in the Material Efficiency variant than in the Clean Technology Scenario.
Efforts from governments, industry and the research community are needed to enable greater uptake of material efficiency. Key actions include: increasing material use data collection and benchmarking; improving consideration of the life-cycle impact in climate regulations and at the design stage; and promoting repurposing, reuse and recycling at end of product and buildings lifetimes.
Executive summary
Clean energy transitions require decoupling of economic growth from material demand
Economic development has historically relied on ever-increasing material demand. However, producing materials consumes resources and energy, resulting in carbon dioxide (CO2) emissions and other environmental effects. Clean energy transitions will affect established material demand trends, through a combination of technology shifts and pursuit of material efficiency strategies. Potential for material efficiency exists throughout value chains, including through designing for long life, lightweighting, reducing material losses during manufacturing and construction, lifetime extension, more intensive use, reuse and recycling. This report examines material efficiency opportunities and implications for three energy-intensive materials – steel, cement and aluminium – and includes deep dives on two major material consuming value chains: buildings construction and vehicles.
Material efficiency can contribute to reducing CO2 emissions. In the Clean Technology Scenario, which aligns with the objectives of the Paris Agreement, material demand is reduced compared to in the Reference Technology Scenario: by 24% for steel (equivalent to about six times the production in the United States in 2017), 15% for cement (two and a half times the production in India in 2017) and 17% for aluminium (1.2 times the primary production in the People’s Republic of China in 2017) in 2060. Material efficiency contributes approximately 30% of the combined emissions reduction for these three materials in the Clean Technology Scenario in 2060.
In the buildings sector, reduced materials demand contributes 10 gigatonnes of cumulative emissions reduction to 2060 in the Clean Technology Scenario, which is a 10% reduction in CO2 emissions from steel and cement use in buildings relative to the Reference Technology Scenario. The demand reduction is largely because of extended buildings lifetimes that are pursued in concurrence with energy efficiency retrofits. In the transport sector, vehicle lightweighting contributes approximately 10% of the global 2060 total passenger light-duty vehicle use-phase emissions reduction in the Clean Technology Scenario relative to the Reference Technology Scenario. This is a substantial portion in the context of the many other emissions reduction strategies such as engine and powertrain efficiency measures and fuel switching (including electrification) being pursued in road vehicles.
Further ambitions on material efficiency can reduce deployment needs for low-carbon industrial process technologies and achieve emissions reduction throughout value chains
Considerable potential exists to push material efficiency beyond the Clean Technology Scenario. The Material Efficiency variant achieves the same degree of energy sector decarbonisation as the Clean Technology Scenario. But it pursues material efficiency strategies to even more ambitious, yet achievable, limits, considering real-world technical, political and behavioural constraints. Strategies pushed considerably further are those more challenging to adopt from the perspective of requiring greater regulatory efforts, stakeholder co ordination, value chain integration, investment, training, shifts in business practices or behavioural change (e.g. improved buildings design and construction, substantial vehicle lightweighting and material reuse). This leads to further material demand reductions compared to in the Clean Technology Scenario, especially for steel (16%) and cement (9%) in 2060. Aluminium use increases (by 5% in 2060) due to vehicle lightweighting outweighing other strategies that put downward pressure on demand.
Material efficiency strategies lead to more moderate deployment needs for low-carbon industrial process technologies for the same CO2 emissions outcome. The Material Efficiency variant achieves the same cumulative industrial emissions as the Clean Technology Scenario, but with a higher emissions intensity for steel (by 4% in 2060) and cement (by 7% in 2060). The emissions intensity of aluminium is somewhat lower (by 9% in 2060). The required cumulative capital investment on low-carbon industrial process technologies is 4% lower by 2060 compared to in the Clean Technology Scenario. For example, cumulative captured and stored CO2 emissions are 45% lower in the cement sector when material efficiency strategies are pursued to such an extent.
Additional material efficiency efforts can achieve emissions reduction beyond the Clean Technology Scenario in some value chains. For example, in the vehicle supply chain, improved fuel efficiency through additional vehicle lightweighting in the Material Efficiency variant reduces net emissions beyond the Clean Technology Scenario by 17% for passenger light-duty vehicles and 9% for light commercial and heavy-duty vehicles in 2060. Total emissions from material production for vehicles increase moderately due to higher production of aluminium, plastics and composites. But this rise is outweighed by emissions savings during vehicle use. In the buildings sector, additional material efficiency efforts relieve pressure on industry without necessarily decreasing buildings use-phase emissions.
Policy and stakeholder efforts are needed to improve material efficiency
Material efficiency does not come without challenges and costs. Real and perceived risks, costs, time constraints, fragmented supply chains, regulatory restrictions and lack of awareness are some of the many barriers to greater uptake of material efficiency strategies. Improving material efficiency will in many cases incur costs, although estimates suggest that these may fall within a reasonable range compared to other emissions mitigations options.
Efforts from all stakeholders will enable greater uptake of material efficiency. Governments and industry can work together to further develop regulatory frameworks and business models in support of material efficiency. Industry can consider the life-cycle impact when designing products and buildings, facilitated by increased data collection and rigorous life-cycle assessment conducted in partnership with researchers. Increasing efforts on end-of-life repurposing, reuse and recycling are also key. Consumers can play a role by increasing demand for material-efficient products that contribute to reducing CO2 emissions.
Findings and recommendations
Policy recommendations
- Increase data collection on material use and the life-cycle impact to set benchmarks and promote best practices.
- Improve consideration of the life-cycle impact in climate regulations to promote material-efficient choices at the design stage.
- Adopt policies that promote durability and long lifetimes to incentivise, for instance, refurbishing and repurposing of buildings instead of demolition.
- Set incentives to reuse and recycle to reduce the need for higher-emission primary materials production, and improve integration of supply chains to facilitate these strategies.
- Shift from prescriptive to performance-based design standards, so that efforts to use materials more efficiently are not unnecessarily restricted.
- Promote education and training programmes on material efficiency
Historical demand trends for materials
Materials are the fundamental building blocks of society. They make up the buildings, infrastructure, equipment and goods that enable businesses to operate and people to carry out their daily activities. They enable services such as transport, shelter and mechanical labour, in many cases through the use of energy.
Global demand for key materials has grown considerably over past decades. Since 1971, global demand for steel has increased by three times, cement by nearly seven times, primary aluminium by nearly six times and plastics by over ten times. Material consumption growth has coincided with population and economic development. In the same period, global population doubled, while global gross domestic product (GDP) grew nearly fivefold.
Although materials bring benefits to society, they are also a source of environmental impact. Converting raw materials into materials for use results in substantial energy consumption and carbon dioxide (CO2) emissions. Along with growth in material demand, energy and emission effects from materials production have grown substantially, by more than one and a half times over the last 25 years. Industry accounted for nearly 40% of total final energy consumption and nearly one-quarter of direct CO2 emissions in 2017.
Demand for materials has grown considerably over past decades. Much of the growth since 2000 has been due to rapid development in the People’s Republic of China (“China”)
Figure 1. Demand growth for key materials, GDP and population
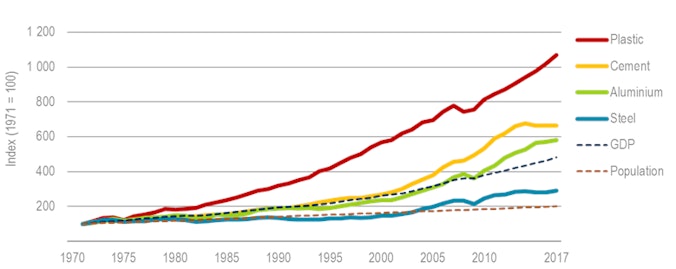
Notes: Outputs of different industrial sectors are displayed on an index basis referred to 1971 levels. Aluminium refers to primary aluminium production only. Steel refers to crude steel production. Plastics include a subset of the main thermoplastic resins. Sources: Geyer, R., J.R. Jambeck and K.L. Law (2017), “Production, use and fate of all plastics ever made”, https://doi.org/10.1126/sciadv.1700782; worldsteel (2018), Steel Statistical Yearbook 2018, www.worldsteel.org/en/dam/jcr:e5a8eda5-4b46-4892-856b-00908b5ab492/SSY_2018.pdf; IMF (2018), World Economic Outlook Database, www.imf.org/external/pubs/ft/weo/2018/01/weodata/index.aspx; USGS (2018a), 2016 Minerals Yearbook: Aluminium, https://minerals.usgs.gov/minerals/pubs/commodity/aluminum/myb1-2016-alumi.pdf; USGS (2018b), 2015 Minerals Yearbook: Cement, https://minerals.usgs.gov/minerals/pubs/commodity/cement/myb1-2015-cemen.pdf; USGS (2017), 2015 Minerals Yearbook: Nitrogen, https://minerals.usgs.gov/minerals/pubs/commodity/nitrogen/myb1-2015-nitro.pdf. Levi, P.G. and J.M. Cullen (2018), “Mapping global flows of chemicals: From fossil fuel feedstocks to chemical products”, https://doi.org/10.1021/acs.est.7b04573.
Industrial total final energy consumption and direct CO2 emissions have grown more than one and a half times over the last 25 years
Figure 2. Global industry final energy consumption and direct CO2 emissions
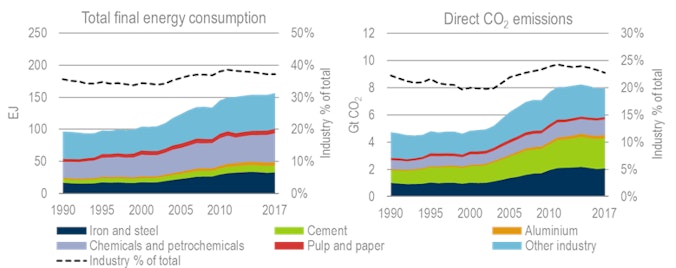
Notes: Industry % of total is industry divided by industry plus non-industrial sectors (including buildings, transport, power generation and heat plants, agriculture, other energy uses and non-energy use). Total final energy consumption includes electricity consumption; direct CO2 emissions do not include indirect emissions from producing the electricity consumed. EJ = exajoules; GtCO2 = gigatonnes of carbon dioxide. IEA 2019. All rights reserved.
Enabling strategies to move towards more sustainable material use
With expected population and economic growth over the coming decades, global demand for steel is expected to increase by approximately 30%, cement by 10% and aluminium by about 75% through to 2060 relative to 2017 levels. This is in the absence of significant changes in the way materials are consumed. The increasing material demand poses challenges for sustainability, including an increase of approximately 15% in CO2 emissions compared to 2017 levels. Therefore, material production and consumption need to be managed.
The Clean Technology Scenario considers substantial reductions in industrial CO2 emissions, which fall by about 45% by 2060 from the 2017 level. While not eliminating the need for strong efforts to reduce emissions intensity of material production, reducing the quantity of materials demanded can contribute to overall emissions reduction, thus reducing deployment needs for low-carbon industrial process technologies for the same CO2 emissions outcome.
These scenarios should not be considered as predictions, but as analyses of the impact and trade-offs of different technology choices and policy targets, thereby providing a quantitative approach to support decision-making in the energy sector.
The Reference Technology Scenario accounts for current country commitments to limit emissions and improve energy efficiency, including nationally determined contributions pledged under the Paris Agreement. By factoring in these commitments and recent trends, this scenario already represents a major shift from a historical “business as usual” approach with no meaningful climate policy response. However, global emissions increase by 8% by 2060 above the 2017 level, which is a pathway far from sufficient to achieve the objectives of the Paris Agreement.
The Clean Technology Scenario lays out an energy system pathway and a CO2 emissions trajectory in which CO2 emissions related to the energy sector are reduced by around three-quarters from today’s levels by 2060. Among the decarbonisation scenarios projecting a median temperature rise in 2100 of around 1.7 1.8 degrees Celsius in the Intergovernmental Panel on Climate Change database, the trajectory of energy- and process-related CO2 emissions of the Clean Technology Scenario is one of the most ambitious in the medium term and remains well within the range of these scenarios through to 2060. The Clean Technology Scenario is the central climate mitigation scenario used in this analysis. It represents a highly ambitious and challenging transformation of the global energy sector that relies on a substantially strengthened response compared with today’s efforts. It opens the possibility of the pursuit of ambitious global temperature goals, depending on action taken outside the energy sector and the pace of further emissions reduction after 2060.
Numerous material efficiency strategies can be applied in the design, fabrication, use and end-of-life stages
Figure 3. Material efficiency strategies across the value chain
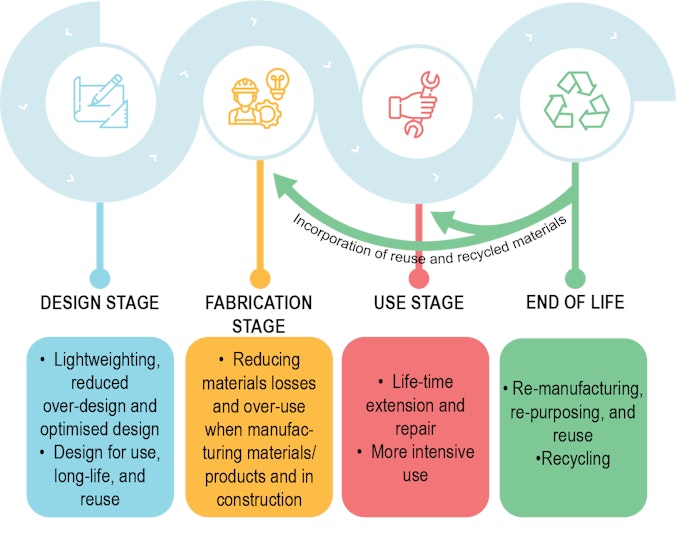
IEA 2019. All rights reserved.
A Material Efficiency variant illustrates the outcome of pursuing material efficiency strategies to their practical yet achievable limits in key value chains, while achieving the same CO2 emissions outcome as the Clean Technology Scenario. Strategies pushed considerably further in the variant are those more challenging to adopt from the perspective of requiring greater regulatory efforts, stakeholder co ordination, value chain integration, investment, training, shifts in business practices or behavioural change. While highly ambitious on material efficiency, the variant remains within real-world technical, political and behavioural constraints.
Different material efficiency strategies can be applied at each stage of supply value chains, including strategies that reduce material demand, those that increase demand for some materials while enabling outweighing CO2 emissions benefits at other stages of the value chain, and those that shift to using lower-emission materials or lower-emission production routes. Some material efficiency strategies interact with each other, leading to synergies in some cases and limitations in others. Key examples of strategies at various stages include the following:
- Design stage – lightweighting and optimisation strategies may enable using fewer materials to provide the same service; designing for long life could result in higher initial material demand but enable outweighing life-cycle emissions savings.
- Fabrication stage – waste and overuse can be reduced when manufacturing materials, during production and in construction; higher-emissions materials can be substituted by lower-emissions materials.
- Use stage – more intensive use and extending product or buildings lifetimes through repair and refurbishment can reduce the need for materials to produce new products.
- End of life – reuse can reduce new materials needs; recycling can enable lower-emission secondary production route
Implications of deploying further material efficiency strategies
Material demand
The Clean Technology Scenario sees considerable divergence from the material demand trends in the Reference Technology Scenario. In 2060, in the CTS, demand is 24% lower for steel (equivalent to about six times the production in the United States in 2017), 15% lower for cement (two and a half times the production in India in 2017) and 17% lower for aluminium (1.2 times the primary production in China in 2017) relative to the RTS.
Considerable potential exists to push material efficiency beyond the Clean Technology Scenario. The Material Efficiency variant achieves the same climate ambitions as the Clean Technology Scenario while pushing material efficiency strategies to highly ambitious yet achievable limits, considering real-world technical, political and behavioural constraints. This leads to further material demand reductions compared to in the Clean Technology Scenario, especially for steel (16% in 2060) and cement (9% in 2060). Aluminium use sees an increase (by 5% in 2060) due to vehicle lightweighting outweighing other strategies that put downward pressure on demand
While material demand grows over time in the RTS, it is considerably reduced in the CTS and MEF
Figure 4. Demand for steel, cement and aluminium by scenario
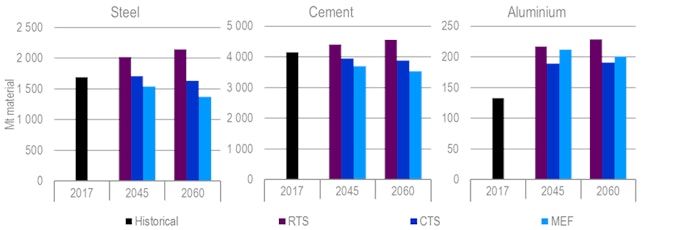
IEA 2019. All rights reserved.
Steel
For steel, the largest cumulative demand reductions from the Reference Technology Scenario to the Clean Technology Scenario occur in the product design and fabrication stage and the use stage, with substantial savings from improving product manufacturing yields and buildings lifetime extension. In the Reference Technology Scenario many buildings would be demolished and rebuilt before the end of their useful life, but major investment in energy efficiency retrofits in the Clean Technology Scenario leads to many of these buildings staying in service longer.
In the Material Efficiency variant, the largest additional savings in steel demand occur from vehicle lightweighting. Significant contributions also come from improving buildings design and construction and reusing steel
There is considerable potential to reduce steel demand at all stages of product and buildings life cycles.
Figure 5. Steel demand change by value chain stage across scenarios in 2060
Cement
Buildings lifetime extension contributes to nearly all of the cement demand reductions in the Clean Technology Scenario relative to the Reference Technology Scenario. This lifetime extension is again the result of retrofits and repurposing pursued in concurrence with buildings energy retrofits.
In the Material Efficiency variant, improvements to buildings design and construction are pursued much more aggressively, contributing to most of the additional reductions beyond the Clean Technology Scenario. The strategies include reducing concrete over-engineering and structural optimisation, promoting concrete steel composite construction, reducing cement content in concrete and reducing on-site construction waste.
The buildings use phase offers the largest potential to reduce cement demand, followed by the design and construction stage
Figure 6. Cement demand change by value chain stage across scenarios in 2060
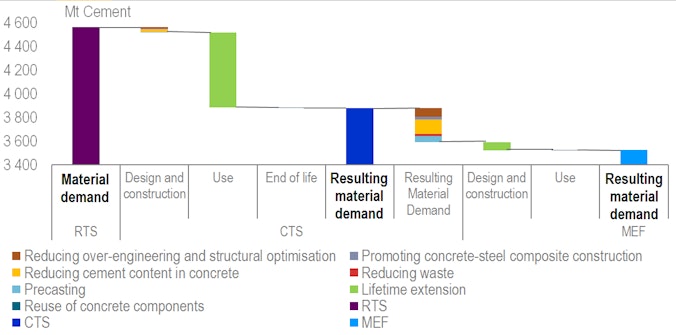
Notes: RTS = Reference Technology Scenario. CTS = Clean Technology Scenario. MEF = Material Efficiency variant. IEA 2019. All rights reserved.
Aluminium
In the Clean Technology Scenario, a considerable downward pressure on aluminium demand occurs because of improved aluminium semi-manufacturing yields and improved product manufacturing yields. However, vehicle lightweighting puts a substantial upward pressure on aluminium demand, as manufacturers substitute aluminium for steel to meet fuel efficiency objectives. The net result is a decline in aluminium demand.
Conversely, in the Material Efficiency variant, additional vehicle lightweighting has the largest effect and results in a net increase in aluminium demand relative to the Clean Technology Scenario. However, a large proportion of the increase from lightweighting is offset by reductions from aluminium reuse.
While reductions in aluminium demand can be achieved at various stages in value chains, a large portion of these reductions is offset by increases in demand from lighter vehicles
Figure 7. Aluminium demand change by value chain stage across scenarios in 200
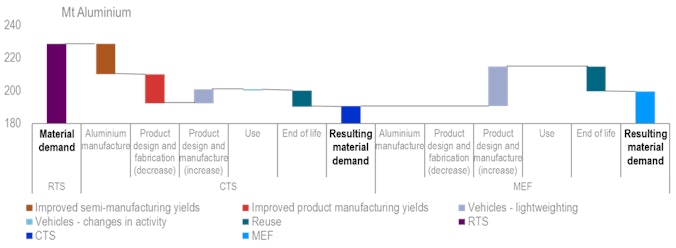
Notes: RTS = Reference Technology Scenario. CTS = Clean Technology Scenario. MEF = Material Efficiency variant. IEA 2019. All rights reserved.
Energy and CO2 emissions
Improving material efficiency can help in achieving emissions reduction, by enabling more moderate deployment of other industry CO2 mitigation levers and by facilitating emissions reduction in other sectors.
In the Clean Technology Scenario, material efficiency assists industry in reducing industrial emissions from the Reference Technology Scenario, contributing approximately 20% of the total emissions reduction for steel, 70% for cement and 30% for aluminium. Material efficiency accounts for approximately 30% of the combined emissions reduction for these three materials in the Clean Technology Scenario in 2060.
Pushing material efficiency further in the Material Efficiency variant leads to more moderate deployment needs for low-carbon industrial process technologies to achieve the same industrial emissions reduction objectives as in the Clean Technology Scenario, particularly when these strategies lead to lower material demand levels. In 2060, the global average direct CO2 emissions intensity of steel production is 4% higher and that of cement is 7% higher in the Material Efficiency variant than in the Clean Technology Scenario, despite achieving the same level of CO2 emissions.
Conversely the global direct CO2 intensity of production of aluminium decreases in the Material Efficiency variant (by 9% in 2060), as the higher material demand requires greater uptake of emission abatement technologies to achieve the same overall emissions levels. This somewhat increased technological effort in the aluminium sector reduces deployment needs for other mitigation options in the transport sector, given that the higher aluminium demand is caused by vehicle lightweighting to reduce transport use-phase emissions.
Lower material demand levels result in higher direct CO2 intensity of production in the MEF while remaining within the CTS industrial emissions level
Figure 8. Direct CO2 and energy intensity of production for steel, cement and aluminium by scenario
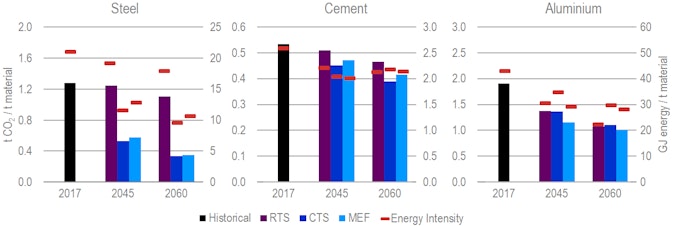
Notes: GJ = gigajoules; t = tonne; tCO2 = tonnes of carbon dioxide. RTS = Reference Technology Scenario. CTS = Clean Technology Scenario. MEF = Material Efficiency variant. IEA 2019. All rights reserved.
Changes in manufacturing direct emissions intensity in the Material Efficiency variant mean that other carbon mitigation technologies need to be deployed at different rates compared to in the Clean Technology Scenario. For steel and cement, lower total material demand means lower cumulative capital technology investment by 2060 in the Material Efficiency variant compared to in the Clean Technology Scenario. For aluminium, the investment is increased. The investment reductions in steel and cement outweigh the increase in aluminium, resulting in a total cumulative technology investment 4% lower in the three subsectors combined. An example of the reduced investment is that cumulative captured and stored CO2 emissions are 45% lower in the cement sector in the Material Efficiency variant than the Clean Technology Scenario.
Instead of reducing deployment needs for low-carbon industrial process technologies while achieving the same decarbonisation levels, material demand reductions could result in additional CO2 emissions reduction. If the Clean Technology Scenario emissions intensity of production were maintained to produce the Material Efficiency variant level of material demand, combined direct emissions in steel, cement and aluminium would be reduced by 7% in 2060 relative to the Clean Technology Scenario. In reality, pushing material efficiency to practical limits would likely result in a combination of reduced industrial emissions and reduced deployment needs for low-carbon industrial process technologies, rather than one or the other only.
Buildings construction value chain
In the buildings sector, material demand in the Reference Technology Scenario increases to over 30% by 2060 above 2017 levels for both steel and cement. This is because rapid construction rates in urban areas coupled with limited efforts to put in place material efficiency strategies sustain recent material demand trends. Steel and cement manufacturing for buildings construction and renovation are responsible for an average of 2.3 gigatonnes of carbon dioxide (GtCO2) annually to 2060, the equivalent of all of India’s emissions in 2017.
In the Clean Technology Scenario, with widespread adoption of buildings codes and standards, demolition rates shrink considerably. Developed countries also implement large-scale deep energy retrofit programmes, which leads to buildings being used for longer. As a result, steel and cement demand are reduced by one quarter in 2060 relative to the Reference Technology Scenario, with buildings lifetime extension contributing over 90% of the reductions.
These material demand reductions lower CO2 emissions from buildings steel and cement use by 10% (10 gigatonnes [Gt]) cumulatively from 2017 to 2060 in the CTS. For steel, material demand reductions account for 16% of the cumulative emissions reduction relative to the Reference Technology Scenario, with the remainder of reductions resulting from changes to lower-emission technologies and process routes to produce steel. For cement, 63% of the emissions reduction is attributable to material demand reduction. While the cumulative reduction in demand for steel and cement is similar (12%), the larger contribution of material demand reduction to reducing cement than steel emissions occurs due to the greater difficulties in decarbonising cement production.
Material demand reductions in the buildings sector help reduce steel and cement emissions in the CTS, while reducing some of the need for material production technology change in the MEF
Figure 9. CO2 emissions related to steel and cement use for buildings construction and renovations by scenario, cumulative from 2017 to 2060
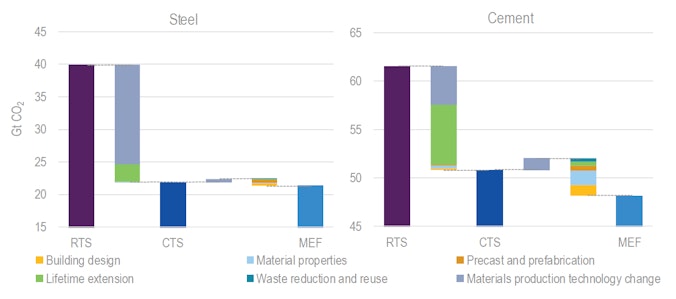
Notes: Emissions from material lost in the semi-manufacturing are not included. RTS = Reference Technology Scenario. CTS = Clean Technology Scenario. MEF = Material Efficiency variant. IEA 2019. All rights reserved.
Pursuing material efficiency strategies to their practical limit in the Material Efficiency variant reduces steel use by an additional 15% and cement use by another 17% in 2060. The additional material demand reductions in the MEF reduce some of the deployment needs for low-carbon materials production process technologies. This results in higher emissions intensity of steel and cement production while still achieving the same carbon budget as in the Clean Technology Scenario. Yet, the steel and cement cumulative CO2 emissions attributable to buildings are lower in the MEF than in the CTS by 5 Gt. This is due to greater reductions in deploying low-carbon industrial process technologies in regions with higher proportions of material demand from end uses other than buildings.
For steel, the largest contributors to material demand reduction in the Material Efficiency variant beyond the Clean Technology Scenario are improvements in buildings design and precasting. Each of these contribute to around 40% of the cumulative emissions reduction attributable to steel demand reduction beyond the Clean Technology Scenario. For cement, improved materials properties (i.e. reducing the cement content in concrete) makes the largest contribution, equal to over one-third of the emissions reduction attributable to cement demand reduction.
Vehicles value chain
For passenger light-duty vehicles, in the Reference Technology Scenario a combination of increasing stocks and lightweighting leads to demand for steel in 2060 that is approximately 20% higher than that in 2017. For aluminium, it is four times higher, and for plastics and composites, it is two times higher. In the Clean Technology Scenario, a combination of reduced vehicle sales, more aggressive lightweighting, improved manufacturing yields and increased reuse results in a considerable reduction in demand for steel (50% lower than in the Reference Technology in 2060), a moderate reduction in demand for aluminium (7% in 2060) and plastics and composites (10% in 2060). The greater push for lightweighting in the Material Efficiency variant results in a further decline in demand for steel (by an additional three-quarters in 2060 relative to the Clean Technology Scenario) and an increase in aluminium (one-quarter in 2060 relative to the Clean Technology Scenario) and plastics and composites (one-third). The material use trends for commercial light-duty and heavy-duty vehicles are similar to those for PLDVs.
Lightweighting – the primary material efficiency strategy pushed further for vehicles in the Material Efficiency variant – results in substantial value chain emissions savings for road vehicles. For passenger light-duty vehicles, lightweighting contributes approximately 10% of the global 2060 total vehicle use-phase emissions reduction in the Clean Technology Scenario over the Reference Technology Scenario, which is a substantial portion in the context of the many other strategies (e.g. modal shifting and fuel switching) that are being pursued in the sector. For commercial light-duty vehicles and heavy-duty vehicles (trucks and buses), lightweighting contributes 3%.
Passenger light-duty vehicle lightweighting leads to net emissions savings in the CTS and additional savings when pushed further in the MEF. Absolute savings in 2060 in the MEF are lower than in 2030, primarily due to increased vehicle electrification, which lowers use-phase emissions savings
Figure 10. CO2 emissions savings from lightweighting throughout the passenger light-duty vehicle value chain by scenario
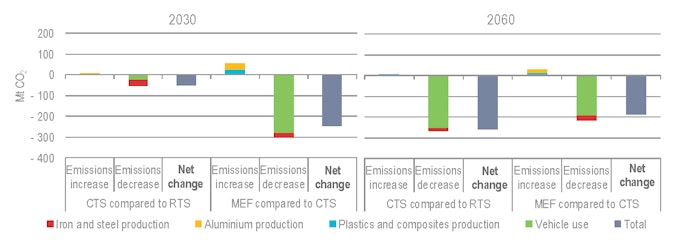
Notes: MtCO2 = million tonnes of carbon dioxide. RTS = Reference Technology Scenario. CTS = Clean Technology Scenario. MEF = Material Efficiency variant. IEA 2019. All rights reserved.
Pushing lightweighting further to its realistic limits leads to additional use-phase emissions reduction in the Material Efficiency variant, equivalent to an additional 20% of Clean Technology Scenario passenger light-duty vehicle use-phase emissions in 2060. While the materials required for this additional lightweighting lead to a moderate increase in emissions for passenger light-duty vehicle material production relative to the Clean Technology Scenario, this is greatly outweighed by the savings in the vehicle use phase. In the Material Efficiency variant, lightweighting results in a net decrease in passenger light-duty vehicle value chain CO2 emissions of 17% in 2060 compared to in the Clean Technology Scenario. Light commercial vehicles and heavy-duty vehicles follow similar trends, with an additional net emissions saving of 9% in 2060 in that value chain.
The absolute CO2 emissions saving in 2060 is about 25% lower than in 2030 in the Material Efficiency variant, despite more aggressive lightweighting. The reason is that a considerable portion of passenger light-duty vehicles have shifted to low-emission fuels, resulting in lower savings potential from lightweighting. While the net change in emissions for battery-electric vehicles depends on the carbon intensity of the electricity grid used to power the vehicle (together with many other factors), in some cases, pushing battery-electric vehicle lightweighting may result in a net increase in value chain emissions. This does not necessarily mean that lightweighting should not be pushed in battery-electric vehicles. Particularly in earlier periods when battery costs are still high, lightweighting could enable larger ranges or lower battery costs, thus facilitating greater uptake of battery-electric vehicles. In later periods, the pressure on increasingly scarce or expensive materials needed to produce batteries may be reduced because lighter vehicles can achieve the same performance (including range) with lighter batteries. For commercial light-duty and heavy-duty vehicles, net absolute emissions savings increase to 2060, as a large portion of these vehicles (particularly trucks) are still running on fossil fuels.
Enabling policy and stakeholder actions
Various challenges need to be overcome to ensure effective use of materials. Without any incentive or requirements to pursue material efficiency, or explicit demand from consumers, designers and manufacturing or construction companies may be unaware of the possible benefits of material efficiency; or they may chose not to pursue material efficiency due to real and perceived risks, financial costs or lost revenues and time constraints. Fragmented supply chains may present challenges for achieving material efficiency, such as when users or demolition contractors are not connected to construction companies to facilitate end-of-life materials reuse. The regulatory environment may also restrict pursuit of material efficiency, such as when prescriptive design standards prevent uptake of new materials or design methods.
Efforts from governments, industry, the research community and society will be needed to overcome these challenges and accelerate the efficient use of materials. Policy and action priorities include the following:
- Increase data collection, life-cycle assessment and benchmarking: more-robust data and analysis on material inputs to end uses and trade-offs throughout value chains related to material inputs and use-phase emissions are needed. This would assist in developing benchmarks, understanding best practices, facilitating optimal decisions in the design stages that consider the life-cycle impact, developing programmes that incentivise material efficiency and adopting mandatory regulations that address the emissions impact of materials.
- Improve consideration of the life-cycle impact at the design stage and in climate regulations: life-cycle impact should be considered at the design stage so that design can help minimise life-cycle emissions. This could be facilitated by expanding the scope of regulations that focus on reducing CO2 emissions in the use phase to cover the full life cycle of products. Life cycle based regulations could incorporate end-of-life requirements to help provide the expected emissions outcomes and standardised life-cycle assessment procedures to reduce the time and cost of compliance.
- Increase end-of-life repurposing, reuse and recycling: extending buildings or product lifetimes through repurposing and refurbishing, aided by government policies promoting durability and long lifetimes, should be prioritised in cases where doing so will not lock in considerably higher use-phase emissions. Greater uptake of reuse and recycling can be facilitated through better integration of supply chains, developing materials inventories, mandating a proportion of reused materials in certain products, expanding the coverage of recycling requirements and requiring producer responsibility.
- Develop regulatory frameworks and incentives to support material efficiency: moving from prescriptive to performance-based standards, including design, health and safety and fire protection standards, would facilitate efficient use of materials while ensuring their intended objectives are achieved. Other government policies to enhance material efficiency include carbon pricing, green certification programmes and government procurement.
- Adopt business models and practices that advance circular economy objectives: integrating policies at the corporate level of businesses can urge decision makers throughout companies to use materials wisely. Planning, monitoring and reporting will promote a culture of material efficiency and deter practices that may increase material use. More-innovative and new business models can also reduce material use, including those that promote a sharing economy and increased digitalisation.
- Train, build capacity and share best practices: material efficiency considerations should be included in education and training programmes for actors throughout value chains. These should include designers, engineers, construction workers, manufacturing companies and demolition companies. Government-supported capacity building would help to ensure compliance when adopting standards that require efficient material use. Best practice sharing among companies would be helpful to promote high standards of material efficiency.
- Shift behaviour towards material efficiency: as consumers, the public can direct demand towards products that are designed and fabricated with material efficiency in mind, and towards sharing economy-focused business models. Material efficient consumer choices at product and buildings end of life are also important. Citizens can vote in support of government policies and investments that aim to reduce carbon emissions, which would aid and accelerate consumer shifts towards material efficiency.
References
Full references for the data behind IEA figures and/or tables featured on this page can be found in the PDF of the full report.
Reference 1
Full references for the data behind IEA figures and/or tables featured on this page can be found in the PDF of the full report.